Description
Introduction
The 2050 Swiss Energy Strategy aims to reduce carbon emissions by embracing renewable energy resources. In Switzerland and worldwide, the heating and cooling sector uses roughly half of the total energy consumed, which is generated primarily by fossil fuels (Quiquerez et al., 2017; Fleuchaus et al., 2018). Aquifer thermal energy storage (ATES) allows low-carbon and/or low-cost heat to be stored until the demand for heat rises, usually in the winter. ATES stores waste heat (e.g. from a power plant, industrial process, or incineration of domestic waste) by injecting hot water into aquifers, and extracting the heat at a later time. ATES is typically operated at moderate temperatures (<25 °C) in thick, unconsolidated aquifers (Fleuchaus et al., 2018). ATES has been shown to be technically and economically successful, and it is becoming widespread in countries such as the Netherlands, which have favorable geologic and legal frameworks (Hartog et al., 2013; Bloemendal et al., 2014; Fleuchaus et al., 2018).
There is interest in expanding ATES to higher temperatures and different reservoir types, like those found in Switzerland. As temperature and pressure increase in ATES systems, the potential for thermo- and poroelastic deformation also increases. We study thermo-hydro-mechanical-economic (THM$) effects in high temperature (HT) (>50 °C) ATES systems (Fig. 1). We hope to understand, mitigate, and avoid ground surface deformation, wellbore integrity problems, hydraulic fracturing, and induced seismicity, which is important due to the proximity that ATES typically has to cities and infrastructure. We also suggest practical engineering guidelines (e.g., optimal well spacing and flow rate) and tools for HT-ATES pre-assessment (i.e., the minimum economically-viable transmissivity).
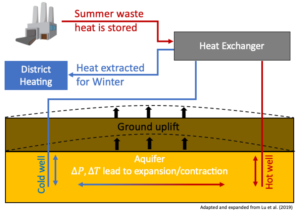
Figure 1: Schematic of THM processes in a HT-ATES system. The temperature and pressure changes lead to mechanical deformations, such as ground uplift.
THM Numerical Model
Our work involves the use of coupled THM numerical models. We use the MOOSE framework to solve the thermo-poro-elastic equations (Gaston et al., 2015; Alger et al., 2019). It is a generalized, parallelized finite element software that facilitates the coupling between different physics. An illustrative example of the numerical model is shown in Figure 2. We collaborate with partners across Europe as part of Geothermcia HEATSTORE. At the national level, we work closely with other universities and industry partners in the Swiss HEATSTORE Consortium. Our models consider input from the energy systems scenario modelers and from the geological understanding at the pilot projects in Geneva and Bern. Likewise, our results inform the experimental design of lab and field work done for these sites.
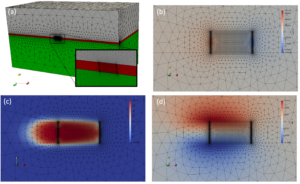
Figure 2: Numerical model results. The 3D mesh (a) uses localized refinement near the wells. The pore pressure (b) and temperature (c) affect the deformation (d).
THM$ Analytical Model
We combine reservoir-engineering with economic calculations in our THM$ approach (Birdsell et al., 2021). We balance three constraints: (a) the size and thermal capacity of the reservoir, (b) avoidance of hydraulic fracturing, and (c) minimization of the levelized cost of heat (LCOH). This provides practical insights on the optimal well spacing, flow rate, and depth for HT-ATES wells. Perhaps more importantly, it gives the minimum economically-viable transmissivity (MEVT), which is the value of reservoir transmissivity that will surely lead to economic infeasability. The MEVT is 5*10-13 m3 and is useful for reservoir pre-assessment at a local or global scale. The THM$ approach is available on Github at: https://github.com/danielbi-ETHZ/THM-Econ.
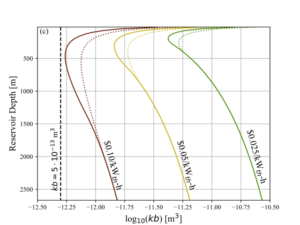
Figure 3: Levelized cost of heat contours as a function of depth and transmissivity. The MEVT is shown by the dashed line.
Related Publications by the GEG Group
REFEREED PUBLICATIONS IN JOURNALS
Birdsell, D. T.,
B. M. Adams, and
M. O. Saar,
Minimum Transmissivity and Optimal Well Spacing and Flow Rate for High-Temperature Aquifer Thermal Energy Storage, Applied Energy, 289/116658, pp. 1-14,
2021.
https://doi.org/10.1016/j.apenergy.2021.116658 [Download] [View Abstract]Aquifer thermal energy storage (ATES) is a time-shifting thermal energy storage technology where waste heat is stored in an aquifer for weeks or months until it may be used at the surface. It can reduce carbon emissions and HVAC costs. Low-temperature ($<25$ \degree C) aquifer thermal energy storage (LT-ATES) is already widely-deployed in central and northern Europe, and there is renewed interest in high-temperature ($>50$ \degree C) aquifer thermal energy storage (HT-ATES). However, it is unclear if LT-ATES guidelines for well spacing, reservoir depth, and transmissivity will apply to HT-ATES. We develop a thermo-hydro-mechanical-economic (THM\$) analytical framework to balance three reservoir-engineering and economic constraints for an HT-ATES doublet connected to a district heating network. We find the optimal well spacing and flow rate are defined by the ``reservoir constraints'' at shallow depth and low permeability and are defined by the ``economic constraints'' at great depth and high permeability. We find the optimal well spacing is 1.8 times the thermal radius. We find that the levelized cost of heat is minimized at an intermediate depth. The minimum economically-viable transmissivity (MEVT) is the transmissivity below which HT-ATES is sure to be economically unattractive. We find the MEVT is relatively insensitive to depth, reservoir thickness, and faulting regime. Therefore, it can be approximated as $5\cdot 10^{-13}$ m$^3$. The MEVT is useful for HT-ATES pre-assessment and can facilitate global estimates of HT-ATES potential.
PROCEEDINGS REFEREED
Birdsell, D., and
M. Saar,
Modeling Ground Surface Deformation at the Swiss HEATSTORE Underground Thermal Energy Storage Sites, Proceedings World Geothermal Congress,
2020. https://doi.org/10.3929/ethz-b-000421353 [Download] [View Abstract]High temperature (>25 °C) aquifer thermal energy storage (HT-ATES) is a promising technology to store waste heat and reduce greenhouse gas emissions by injecting hot water into the subsurface during the summer months and extracting it for district heating in the winter months. Nevertheless, ensuring the long-term technical success of an HT-ATES project is difficult because it involves complex coupling of fluid flow, heat transfer, and geomechanics. For example, ground surface deformation due to thermo- and poro- elastic deformation could cause damage to nearby infrastructure, and it has not been considered very extensively in the literature. The Swiss HEATSTORE consortium is a group of academic and industrial partners that is developing HT-ATES pilot projects in Geneva and Bern, Switzerland. Possible target formations at the Geneva site include: (a) fractured Cretaceous limestone aquifers interbedded within lower-permeability sedimentary rock and (b) Jurassic reef complex(es), also potentially fractured. In this work we offer numerical modeling support for the Geneva site. A site-specific, hydro-mechanical (HM) model is created, which uses input from the energy systems scenarios and 3D static geological modeling performed by other Swiss consortium partners. Results show that a large uplift (> 5 cm) is possible after one loading cycle, but a sensitivity analysis shows that uplift is decreased to ≤ 0.3 cm if the aquifer permeability is increased or an auxiliary well is included to balance inflow and outflow. Future work includes running coupled thermo-hydro-mechanical (THM) models for several loading and unloading cycles. The THM framework can help inform future decisions about the Swiss HT-ATES sites (e.g. the final site selection within the Geneva basin, well spacing, and operating temperature). It can also be applied to understand surface deformation in the context of geothermal energy, carbon sequestration, and at other ATES sites worldwide.
Guglielmetti, L.,
P. Alt-Epping,
D. Birdsell,
F. de Oliveira,
L. Diamond,
T. Driesner,
O. Eruteya,
P. Hollmuller,
et al., and
M.O. Saar,
HEATSTORE SWITZERLAND: New Opportunities of Geothermal District Heating Network Sustainable Growth by High Temperature Aquifer Thermal Energy Storage Development, World Geothermal Congress,
2020. [View Abstract]HEATSTORE is a GEOTHERMICA ERA-NET co-funded project, aiming at developing High Temperature (~25°C to ~90°C) Underground Thermal Energy Storage (HT-UTES) technologies by lowering the cost, reducing risks, improving the performance, and optimizing the district heating network demand side management at 6 new pilot and demonstration sites, two of which are in Switzerland, plus 8 case studies. The European HEATSTORE consortium includes 24 contributing partners from 9 countries, composing a mix of scientific research institutes and private companies.
The Swiss consortium, developing HEATSTORE in Switzerland, involves of two industrial partners (Services Industriels de Geneva - SIG and Energie Wasser Bern - EWB) and four academic partners (Universities of Geneva, Bern, Neuchâtel and ETH Zurich), with support from the Swiss Federal Office of Energy. The aims are to develop two demonstration projects for High Temperature Aquifer Thermal Energy Storage (HT-ATES) in the cantons of Geneva and Bern such that industrial waste heat can be converted into a resource.
This paper presents the results of the first year of activities in the Swiss projects. The activities planned cover subsurface characterization, energy system analysis, surface implementation design, legal framework improvement and business modelling to ensure the sustainability of the projects. This approach is supported by large industrial investments for subsurface characterization. Two wells, down to 1200m below surface level (bsl) are being drilled in the Geneva area to tap potential targets in the carbonate Mesozoic units and at least three additional wells, down to 500m bsl will target the Molasse sediments in the Bern area next year. These wells allow subsurface exploration and characterization and will provide data, used for detailed THMC modelling to assess the thermal energy storage potential at the two sites in Switzerland. The results of such numerical modelling are combined with energy system analysis to quantify the waste heat availability and heat demand and hence optimize the production and injection operations. The outcomes of the coupled assessments will aid in designing the integration of the new installations into the district- heating network. Legal framework improvements, based on complete technical evaluation and on the best-practice sharing with the other European partners, will be an enabling tool to accelerate the implementation of the HT-ATES systems, while business modelling helps calibrate the economic feasibility of the projects and helps industrial partners to plan future investments.
Other References
Alger B., Andrš D., Carlsen R. W., Gaston D.R., Kong F., Lindsay A. D., Miller J. M., Permann C. J., Peterson J. W., Slaughter A. E., and Stogner R.: MOOSE Web Page. https://mooseframework.org. (2019).
Bloemendal, M., Olsthoorn, T., & Boons, F.: How to achieve optimal and sustainable use of the subsurface for Aquifer Thermal Energy Storage. Energy Policy, 66, (2014), 104-114.
Fleuchaus, P., Godschalk, B., Stober, I., and Blum, P.: Worldwide application of aquifer thermal energy storage–A review. Renewable and Sustainable Energy Reviews, 94, (2018), 861-876.
Gaston, D. R., Permann, C. J., Peterson, J. W., Slaughter, A. E., Andrš, D., Wang, Y., … and Zou, L.: Physics-based multiscale coupling for full core nuclear reactor simulation. Annals of Nuclear Energy, 84, (2015), 45-54.
Hartog, N., Drijver, B., Dinkla, I., & Bonte, M.: Field assessment of the impacts of Aquifer Thermal Energy Storage (ATES) systems on chemical and microbial groundwater composition. Proceedings, European Geothermal Conference, Pisa, Italy (2013).
Quiquerez, L., Lachal, B., Monnard, M., & Faessler, J. (2017). The role of district heating in achieving sustainable cities: Comparative analysis of different heat scenarios for Geneva. Energy Procedia, 116, 78–90. https://doi.org/10.1016/j.egypro.2017.05.057